Since the discovery of hydrothermal vents 40-years ago, long-term time-series have focused on mid-ocean ridge systems. Based on these studies, hydrothermal vents are widely considered to be dynamic, ephemeral habitats. Under this premise, national, and international regulatory bodies are currently planning for the commercial mining of polymetallic sulfide deposits from hydrothermal vents. However, here we provide evidence of longevity and habitat stability that does not align with historic generalizations. Over a 10-year time-series focused on the back-arc basin systems off the west coast of the Kingdom of Tonga (South Pacific), we find the hydrothermal vents are remarkably stable habitats. Using high-resolution photo mosaics and spatially explicit in situ measurements to document natural changes of five hydrothermal vent edifices, we discovered striking stability in the vent structures themselves, as well as in the composition and coverage of the vent-associated species, with some evidence of microdistribution permanence. These findings challenge the way we think about hydrothermal vent ecosystems and their vulnerability and resilience to deep-sea mining activities.
Introduction
Hydrothermal vents create structures of metal-rich deposits while providing energy for chemoautotrophic primary production, which supports dense assemblages of vent-associated fauna. These submarine features are globally distributed along mid-ocean ridges, back-arc basin spreading centers, volcanic arcs, and intra-plate volcanoes (Baker and German, 2004; Beaulieu, 2015). Since their discovery 40-years ago, it has proved difficult to mount expeditions with sufficient temporal resolution to study successional processes at hydrothermal vents (Glover et al., 2010). Long-term time-series have been achieved at only a handful of the better-known sites, the majority of which are on medium to fast spreading rate mid-ocean ridges (≥55 mm·year−1), such as the Galapagos Rift, the East Pacific Rise, and the Juan de Fuca Ridge (Glover et al., 2010; Beaulieu, 2015). Based on these studies, hydrothermal vents are widely considered to be dynamic, ephemeral habitats (Goldfarb et al., 1983; Chevaldonné et al., 1991; Haymon et al., 1993; Sarrazin et al., 1997; Tunnicliffe et al., 1997; Shank et al., 1998; Johnson et al., 2000; Mullineaux et al., 2003; Cowen et al., 2007; Sen et al., 2014). However, the long-term natural variability of back-arc basin hydrothermal vents is largely unstudied (Boschen et al., 2013; Levin et al., 2016). With the emerging deep-sea mining industry focused on South Pacific back-arc basin hydrothermal systems, this lack of baseline data is a major impediment to informed decision-making. In the interim, the Kingdom of Tonga, Fiji, Papua New Guinea, and the Solomon Islands have all granted licenses for mining exploration of polymetallic sulfide deposits (also known as seafloor massive sulfide deposits) in their waters, sovereign states are investing in their own mining programs (e.g., Japan), and the International Seabed Authority (ISA) has issued contracts for mining exploration in the high seas (Beaulieu, 2015; Levin et al., 2016; Shirayama et al., 2017).
It has been said that the environmental consequences of active hydrothermal vent mining activities could be similar to natural disturbances and the associated ecosystem recoveries, such as the destruction of structures and mortality of associated fauna (Boschen et al., 2013; Van Dover, 2014; Levin et al., 2016; Gollner et al., 2017). However, this assumption relies on the premise that vent species live within highly dynamic or ephemeral habitats and are adapted to, or at least tolerant of, a high pace of natural variability and so will be resilient (SPS, 2013; Van Dover, 2014).
While national regulatory bodies and the ISA are currently drafting exploitation regulations for deep-sea mining and the measures for environmental protection (Van Dover et al., 2017), the generalized premises that underpin the anticipated impacts and the potential recovery from mining activities require scrutiny. For example, a major premise of deep-sea mining was recently demonstrated unreliable when researchers found that nearby vents (75 km apart) did not support the same vent-associated communities (Goffredi et al., 2017), corroborating evidence that patchwork mining extraction will not necessarily avoid a loss of vent-associated biodiversity (Van Dover et al., 2017). Because mining will be proceeding in back-arc settings (e.g., Nautilus Minerals Niugini Ltd “Solwara 1 Project”; CNS, 2008), it is important to examine the inference that back-arc basin vent communities show the same potential for resilience to disturbance as the better-known mid-ocean ridge vent communities (i.e., ability to maintain structure and function or to recover structure and function quickly). To test this null hypothesis of little to no difference between these systems in this regard, we repeatedly surveyed five study sites on the side of active edifices in the Lau Basin over 10 years to resolve the natural rates of structural and functional changes experienced at back-arc basin hydrothermal vents.
Methods
Study Sites
Our study sites are located within the Lau Basin, at the Tow Cam, ABE, and Tu'i Malila vent fields (Figure 1A; Table 1) (TC1C, ABE1-3C, and TM1C). These vent fields stretch along >200 km of the Eastern Lau Spreading Center and Valu Fa Ridge, with spreading rates of ~55–80 mm·year−1(Mottl et al., 2011; Beaulieu, 2015).
FIGURE 1
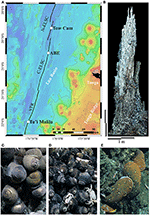
Figure 1. The environmental settings of the study. (A) Our study sites are located within the Tongan region of the Lau Basin, along the North and Central Eastern Lau Spreading Center and the Valu Fa Ridge. The five hydrothermal vent edifices are within the Tow Cam, ABE, and Tu'i Malila vent fields. These vent fields are all located within deep-sea mining license blocks (see text for details). (B) This example high-resolution photo mosaic (ABE1C, 2016) shows the visual distinction between the lighter/white anhydrite summit deposits and the darker/grayish-brown sulfide base deposits, as well as the aggregated clusters of vent-associated fauna on the sulfide surfaces. The edifice sulfide deposits host three faunal groups of chemoautotrophic bacteria-containing invertebrates, (C) the provannid snails Alviniconcha spp. and (D) Ifremeria nautilei, and (E) mussel Bathymodiolus septemdierum.
TABLE 1
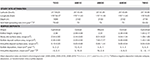
Table 1. Attributes of the long-term study sites on five active hydrothermal vent edifices in the Tow Cam (TC), ABE, and Tu'i Malila (TM) vent fields, and changes over time.
All five study sites are within deep-sea exploration tenements currently licensed to Nautilus Minerals Inc (which require renewal in 2019; pers comm Alison Swaddling). Within these tenements, the ABE and Tu'i Malila vent fields are specifically identified as mining prospect sites (Jankowski, 2012). In addition to the contiguous Nautilus Minerals tenements spanning the spreading centers (Jankowski, 2012), Kiost Minerals (South Pacific) Ltd has been granted exploration tenements between those granted to Nautilus Minerals and the Tonga Ridge (pers comm Alison Swaddling) (Figure 1A).
Deep-Sea Expeditions
Our research expeditions took place in September 2006, May-June 2009, and April-May 2016. Fieldwork was carried out using the remotely operated vehicle (ROV) Jason II from aboard the research vessel (R/V) Melville and from aboard the R/V Thomas G. Thompson in 2006 and 2009, and the ROV ROPOS from aboard the R/V Falkor in 2016. While remote sensing of the geomorphology of vent fields and the geochemistry of hydrothermal plumes can provide some evidence of stochastic geological events, investigating the natural variability in structure and function of vent habitats requires in situ time-series of the vents and associated fauna. During each expedition, we used high-resolution (sub-centimeter) photo mosaics (Figure 1B) and spatially explicit in situ measurements to document changes in the structural deposits, hydrothermal fluid, and vent communities. We imaged the edifices with a forward-mounted camera and made spatially explicit in situ thermal measurements using a temperature probe and the ROV manipulators. Details on the hardware used before 2016 are available in Podowski et al. (2010) and Sen et al. (2014). During the 2016 expedition, we used an add-on 18-megapixel Canon EOS Rebel T5i digital still camera with a 20 mm Canon lens and the ROPOS high-temperature probe. Although equipment varied between expeditions, we regard the differences as negligible since image resolution was consistently sub-centimeter and there was little to no variability in ambient seawater measurements between years (Table 2).
TABLE 2
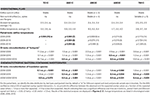
Table 2. Spatio-temporal characteristics and analyses of the hydrothermal fluids and chemoautotrophic bacteria-containing invertebrates (foundation species) at the long-term study sites: Tow Cam (TC), ABE, and Tu'i Malila (TM) vent fields.
The collection, processing, and digitization of images and in situ thermal measurements followed procedures initially outlined in Podowski et al. (2009, 2010), with adaptations for the vertical orientation of edifices outlined in Sen et al. (2013, 2014). For hydrothermal vent research, it is often necessary to consolidate data from a series of discrete cruises that were not originally designed for temporal studies (Glover et al., 2010). In contrast, our long-term, high-resolution time-series was designed for repeated surveys. To replicate the surveys, repositioning with the same coordinates, depth, and heading relative to deployed physical markers, was crucial to locate the study sites, minimalize the effect of parallax, and enable paired statistical comparisons of replicated measurements (Sen et al., 2014).
Geographic Information Systems (GIS) Data
Using a house-painting photo acquisition technique and a customized large-area mosaicing Matlab program (Pizarro and Singh, 2003), we generated a high-resolution photo mosaic of one side of each edifice, for each expedition. Mosaics were georeferenced and digitized in ArcMap 9.0 and 10.3. To map the physical structure of the edifices, we digitized a vector layer (area polygon) for the two main edifice substrates, sulfide and anhydrite deposits, which are visually distinct with different physical characteristics (Goldfarb et al., 1983; Haymon, 1983; Tunnicliffe and Juniper, 1990), and a point layer for the temperature measurements and vent orifice locations. We also digitized vector layers for the three local faunal groups of chemoautotrophic bacteria-containing invertebrates, the provannid snails Alviniconcha spp. (cryptic species: A. kojimai, A. boucheti, A. strummeri; Johnson et al., 2015) and Ifremeria nautilei, and the mussel Bathymodiolus septemdierum (prior epithet: B. brevior; Breusing et al., 2015) (Figures 1C–E). These primary producers and foundation species are community-structuring species and hence are the focus of our investigation concerning the natural stability of biological processes and ecosystem function on the edifices. For the substrate vectors, we inferred that the obscured edifice beneath clusters of foundation species were sulfide deposits since these molluscs avoid anhydrite surfaces (Sen et al., 2013).
Statistics
We performed statistical analyses in R-3.2.2. Our temporal analyses included sub-decadal and decadal scales (n = 3), based on the intervals between the expeditions: 2 years 8 months, 6 years 11 months, and 9 years 7 months (~3, 7, and 10 years). For the thermal data, we used paired t-tests (parametric) and Wilcoxon signed-rank tests (non-parametric), for a total of 13 tests (temperature measurements were not collected for TC1C in 2016). To assess the temporal variability of the faunal groups, we ran a Friedman test for each faunal group using the edifices as replicates, followed by three paired tests to investigate each temporal interval separately (i.e., non-parametric one-way ANOVAs and paired t-tests or Wilcoxon signed-rank tests).
Spatio-Temporal Analysis
To assess the spatio-temporal variability on each edifice, we tested for spatial overlap over time. We ran two sets of these analyses, one for the microdistribution of “hotspots” and one for the microdistribution of foundation species. We used surficial anhydrite as a visual proxy of “hotspots” since it precipitates on surfaces experiencing hydrothermal fluid flow >150°C (Bischoff and Seyfried, 1978). We consolidated the foundation species for this analysis because the three faunal groups have substantial overlaps in realized niches (Podowski et al., 2010) and often occur commingled. Using ArcGIS, we identified areas of intersection (∩) between surveys at the sub-centimeter resolution of the images and calculated the percent of spatial overlap as a function of the microdistribution in the later survey. We also calculated the expected spatial overlap assuming each year's microdistribution was by chance, independent and random, based on the percent of edifice area and the addition rule of probability. To test for a significant difference between the observed and the expected overlap, we used bootstrapped 95% confidence-interval (CI) tests (with 999 iterations). The inverse of the CI test indicates if there was a significant change in the microdistribution (H1). We interpreted results as one of two spatio-temporal patterns, either redistribution occurred, randomly or to new areas (H1: observed overlap was not significantly greater than expected), or the microdistribution was spatially stable (H0: observed overlap was significantly greater than expected).
Results and Discussion
Hydrothermal Vent Fields
Despite the spatial and temporal extent of our surveys (Figure 1A), our research was not affected by large-scale stochastic geological events over the 10-year study period. While the processing of bathymetry data is ongoing, preliminary analyses of bathymetric surfaces and visual observations of the seafloor indicate that no significant changes in surface geology occurred over the decade (pers comm Vicki Ferrini). Here we document that three medium spreading rate back-arc basin vent fields have provided stable hydrothermal environments for over a decade, with no evidence of regional disruption from sub-surface hydrothermal activity or catastrophic eruptions or lava flows. These findings support the hypothesis that, unlike the well-studied dynamic mid-ocean ridge systems, the associated communities of these vent fields need not be as naturally resilient to the same fast-paced large-scale disturbance regime. In comparison, mid-ocean ridge time-series have shown catastrophic events occurring at interannual to decadal-scales, with system dynamics positively correlated with the ridge spreading rate (Haymon et al., 1993; Johnson et al., 2000; Cowen et al., 2007; Glover et al., 2010).
Edifices
The sides of the edifices surveyed ranged from 1.64 to 4.90 m in height and 0.54 to 9.22 m2 in area (Table 1; Figure 2A). All five edifices were predominantly sulfide deposits. While the anhydrite spires were prone to cycles of growth and collapse or dissolution (cf. Tunnicliffe and Juniper, 1990), in comparison, the sulfide deposits were physically stable between surveys. New sulfide deposition was slow, and the general shapes and sizes of the edifices did not change over the decade. As previously mentioned, time-series data is limited, but the general model is that hydrothermal vent system dynamics are positively correlated with the ridge spreading rate. However, the sulfide areas of our Lau Basin edifices grew by 2.7 ± 1.3%·year−1, an average rate comparable to that documented at a slower spreading center (3%·year−1 at Smoke and Mirrors on the Juan de Fuca Ridge, 56 mm·year−1; Sarrazin et al., 1997; Beaulieu, 2015). Although deposition rates can slow from clogging or reaching a growth-weathering equilibrium as edifices mature (Goldfarb et al., 1983; Haymon, 1983), after an adjacent ABE edifice was toppled in 2009, there was no difference between its new and its previous “mature” deposition rates (unpublished ABE4C data: 2 cm2·year−1 before and after). These growth rates are much slower than the rapid formation of edifices documented on mid-ocean ridges with similar spreading rates (e.g., 13°N East Pacific Rise, 91 mm·year−1; Hekinian et al., 1983; Beaulieu, 2015). Using their constant sub-decadal sulfide deposition rates (Table 1), we can estimate it took ABE3C ~22 years and TM1C ~40 years to solidify into the still relatively small edifices observed in 2016. Following a mining event, these slow sulfide deposition rates could further hinder recovery through slow reversibility of the substrate removal and habitat loss (Gollner et al., 2017), decreasing the resilience of the back-arc basin vent-associated communities.
FIGURE 2
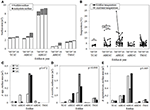
Figure 2. The natural changes in (A) the size of the edifices and the two substrate types, (B) the fluid temperatures, and (C–E) the three chemoautotrophic bacteria-containing invertebrates for the five edifices over the study decade (2006, 2009, 2016). Lines show the pairings between repeated temperature measures and asterisks indicate a significant difference over time. A set of three bars show repeated faunal measures, but unlike with the temperature, there were no significant changes over time (Friedman tests where n = 5 edifices). For summarized statistics, see Tables 2, 3.
Venting
On four of the five edifices, the number and locations of orifices venting hydrothermal fluid did not change between years (visual observation of shimmering or discolored fluid) (Table 2). On the exception edifice, TM1C, three of the six orifices were stable. The distribution and intensity of hydrothermal flow, detected with spatially explicit in situ temperature measurements, was also remarkably stable on all five edifices at the sub-decadal and decadal scales (Figure 2B; Table 2). Over the study decade, three of the five edifices showed no significant change in temperature among paired measurements, while the variability on the other two edifices was relatively small (differences ≤0.6 and ≤2.6°C; Figure 2B). Subsurface mixing dilutes vent fluids with cooler water, and our point measurements ranged from ambient at 2.3–2.5°C to 34.4°C (Figure 2B). That said, small regions of focus flow at Lau Basin hydrothermal vents can be in excess of 350°C (Fouquet et al., 1991). To document this spatially-finite higher temperature range, we used surficial anhydrite precipitate as a visual proxy of “hotspots” >150°C (Bischoff and Seyfried, 1978). Over the majority of temporal-scales investigated, the microdistribution of “hotspots” was markedly stable, with clear spatial patterns of permanence on each edifice (e.g., the top-right corner of TC1C, the summit spires of ABE1C) and up to 50% precise overlap at sub-decadal scales (significantly greater overlap than what is expected to have occurred by chance) (Figure 3A). These multiple lines of evidence indicate a stability in hydrothermal venting on the outer portions of Lau Basin edifices that is different from vent fields on mid-ocean ridges of similar spreading rates (e.g., 13°N East Pacific Rise and Smoke and Mirrors on the Juan de Fuca Ridge, 91 and 56 mm·year−1respectively; Fustec et al., 1987; Sarrazin et al., 1997; Beaulieu, 2015).
FIGURE 3
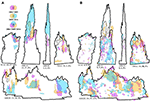
Figure 3. The five hydrothermal vent edifices with the microdistribution of (A) “hotspots” between years (≥150°C) and (B) foundation species between years. The colored polygons represent the microdistributions observed during each survey: pink in 2006, orange in 2009, and blue in 2016. Thick black lines denote the study sites' boundaries in 2016, while thin black lines denote previous years' boundaries (i.e., edifice growth). The three values provided underneath each edifice are the percent of spatial overlap in the microdistribution between years (i.e., spatial intersection, ∩), where bolded values denote a statistical change in the microdistribution. For detailed statistical outputs, including the differences between observed and expected overlap, see Table 2.
Without focused high-temperature flow measurements, we cannot directly speak to the stability of the end-member fluid (i.e., uncontaminated hydrothermal fluid). However, as the source of the diffuse hydrothermal venting, we would expect the end-member fluid temperature to be equally or more stable over the monitoring period than our time-series (cf. Tivey et al., 2002). Ten-year results of little to no natural change in the end-member chemical composition have been documented in the Mariana back-arc basin (Alice Springs, 32 mm·year−1; Beaulieu, 2015; Ishibashi et al., 2015). In the present study, at least some stability in the end-member fluid chemical composition is indirectly evident from observations of consistent edifice deposition (hydrothermal fluid precipitate) and the realized distributions of vent-associated fauna.
Vent-Associated Fauna
The realized distribution of the three study faunal groups reflects their requirement for reduced substrates, their temperature and chemistry tolerances, responses to biological interactions, and the physical stability of the substrate (Henry et al., 2008; Podowski et al., 2009, 2010; Sen et al., 2013). It then follows that the unusual habitat stability of the Lau Basin edifices would be expected to reflect similarly high stability in the composition, coverage, and microdistribution of the foundation species at the same temporal scales. That said, hydrothermal vents are not static systems and any habitat stability experienced would be the result of dynamic systems in equilibrium. All five study sites were inhabited by I. nautilei and B. septemdierum in 2006, 2009, and 2016 (Figures 2C–E). I. nautilei was the dominant megafauna on the edifices (≤1.95 m2) and experience the largest changes in coverage, B. septemdierum was also abundant (≤0.80 m2), while A. spp. were relatively sparse if present at all (≤0.40 m2).
The coverage of each faunal group between years was remarkably constant, with no significant changes at the sub-decadal and decadal temporal scales (Figures 2C–E; Table 3). Although not significantly different, variability was observed, with several notable peaks or dips in coverage. Of the five edifices, ABE2C experienced the largest changes in coverage, with relatively less A. spp. in 2006 and more I. nautilei but less B. septemdierum in 2009. The peaks in coverage of the already dominant I. nautilei on ABE1C in 2016 and ABE2C in 2009 support the hypothesis that I. nautilei outcompetes A. spp. for new habitat when both are already locally established (Sen et al., 2014). In addition, there were no changes in the presence of anhydrite-hosted megafauna on any edifice between years (a sparse community dominated by higher trophic level palm worms, scale worms, and shrimps; Sen et al., 2013). Our results indicate stability in the composition and coverage of the foundation species on Lau Basin edifices that is contrary to time-series studies at vents of similar medium-spreading rates (e.g., Rose Garden on the Galapagos Ridge, 63 mm·year−1; Hessler et al., 1988; Beaulieu, 2015). Comparable levels of decadal-scale stability have only been previously described for slow-spreading sites on the Mid-Atlantic Ridge (Eiffel Tower and TAG mound, 20 and 24 mm·year−1; Copley et al., 2007; Cuvelier et al., 2011; Beaulieu, 2015). Increasing the frequency of disturbances to hydrothermal vent habitats through mining activities has the potential to change the ecological balance (e.g., favorable conditions for “weedy” r-selected species, rather than long-lived K-selected species) and cause vent-endemic species extinctions and unforeseeable changes in ecosystem structure and function (Van Dover, 2014; Levin et al., 2016).
TABLE 3
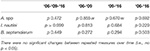
Table 3. The statistical results for repeated measures of chemoautotrophic bacteria-containing invertebrate coverage on the edifices (n = 5): Friedman tests for all years combined and three sets of paired tests (paired t-test by default or “w” for Wilcoxon signed-rank test).
Since it is unknown when they were established, we are limited in reporting only that these assemblages have persisted for at least 10-years on each edifice, although it is likely they have inhabited them for longer. There is no evidence of the community succession expected to occur following an increase or decrease in vent activity (i.e., a nascent or cessation event) described by Sen et al. (2014). Because of adverse weather during the 2016 expedition, we were unable to re-survey long-term study sites in the Kilo Moana or Mariner vent fields. This is particularly unfortunate because Kilo Moana may be experiencing waning hydrothermal activity (Sen et al., 2014), providing an opportunity to document the rate of a natural disturbance and succession event.
Faunal Microdistribution
The three study faunal groups, which often aggregate because of overlapping niches (Podowski et al., 2009) (Figure 1B), are mobile molluscs that require exposure to vent fluid for their sustenance (Podowski et al., 2009, 2010; Sen et al., 2013). They can move in response to changes in local environmental gradients caused by dynamic underlying processes such as variability in surface and sub-surface micro-plumbing, fluid properties, and bacterial communities (Goldfarb et al., 1983; Chevaldonné et al., 1991; Sarrazin et al., 1997; Shank et al., 1998; Mullineaux et al., 2003; Sen et al., 2014). As sulfide deposits slowly solidify, new inhabitable substrate with exposure to hydrothermal vent fluids is formed (Goldfarb et al., 1983; Haymon, 1983). Despite their mobility and the potential variability of environmental gradients, here we document several examples of remarkable permanence in the distribution of foundation species on Lau Basin edifices between years (Figure 3B; Table 2).
As expected, higher deposition rates corresponded to the decreased spatial stability of foundation species (ABE1C and 3C; Table 2; Figure 3B), yet at least one location on each edifice was occupied by an aggregation of foundation species in 2006, 2009, and 2016. Edifices that were more densely populated by foundation species showed the clearest spatial patterns of permanence (e.g., the bottom-left corner and summit aggregations on TM1C, the center and right-side aggregations on ABE2C). On three of the edifices, the microdistribution of the foundation species was markedly stable over one or more temporal scales, with 9–50% precise overlap between years (Figure 3B). TC1C and TM1C demonstrated sub-decadal spatial stability, while ABE2C demonstrated sub-decadal and decadal spatial stability (significantly greater overlap than what is expected to have occurred by chance). During the 2016 expedition, we revisited each site 3 hours to 14 days after the main survey. There was little to no change in the distribution of the aggregations evident at these finer temporal scales of hours to days (resolved to ~5 cm). Considering how long some vent species live, it is plausible that individual organisms have inhabited the same locations on the Lau Basin edifices for over ten years (e.g., B. septemdierum live >18 years; Schöne and Giere, 2005). The cumulative evidence of the slow pace of natural disturbance and variability experienced by the La Basin foundation species indicates the vent-associated community will be less resilient to anthropogenic disturbances than anticipated, and that remediation as a strategy to address the loss of biodiversity at and around a back-arc basin mine site is likely unrealistic (Van Dover et al., 2017).
Conclusion
This novel long-term time-series study in a back-arc basin presents a quantitative structural and functional assessment of the natural habitat variability of active hydrothermal vent edifices along medium-rate spreading centers. Here we discovered striking stability in the physical and thermal conditions, as well as in the composition, coverage, and microdistribution of vent-associated species, which contrasts the widely accepted narrative that hydrothermal vents are highly dynamic and ephemeral. This evidence opposes a major premise underpinning the deep-sea mining industry, indicating that back-arc basin vents and their communities experience slower natural changes and a lower frequency of natural disturbance than mid-ocean ridge systems with similar spreading rates, and will therefore be less resilient to anthropogenic disturbances than anticipated (through slow reversibility of the habitat loss and long-lived inhabitants that may not be adapted to, or tolerant of, a high pace of natural variability).
With the imminence of industrial mining in back-arc basins, it is important new discoveries be incorporated into the national and international environmental regulations that will govern deep-sea mining (e.g., predicting and mitigating the effects of mining activities and recovery monitoring plans). Our findings support the assessment that efforts toward mitigating hydrothermal vent biodiversity loss should focus on avoiding and minimizing harm (Van Dover et al., 2017).
This evidence of overall longevity and habitat stability at back-arc basin hydrothermal vents does not align with historic generalizations, challenging the way we think about vent ecosystems and highlighting the need for time-series studies of hydrothermal vents in a broader scope of tectonic and volcanic settings. We propose that this level of structural and functional stability is not unique in back-arc basins or the different hydrothermal vent settings, but that the publication bias for time-series data obtained in response to major volcanic eruptions and a historic research bias for the better-known mid-ocean ridge sites (Glover et al., 2010) has perpetuated a distorted generalized narrative. In moving forward, it will be crucial to determine whether our study encompassed an anomalous time-period on anomalously stable spreading centers, or if this level of stability is common within back-arc basins.
Author Contributions
CF initiated and attained funding for the decadal research project, and supervised the research and writing. CD conceived, designed and performed the analyses for this research, and wrote the manuscript. Both authors discussed the results and implications and worked on the manuscript at all stages.
Conflict of Interest Statement
The authors declare that the research was conducted in the absence of any commercial or financial relationships that could be construed as a potential conflict of interest.
Acknowledgments
We gratefully acknowledge all those who contributed to the success of the Lau Basin monitoring program (2005-2016). Fieldwork was made possible by the expertise of the crews of the R/V Melville, the R/V Thomas G. Thompson, and the R/V Falkor and the crews of Jason II and ROPOS. We thank Arunima Sen, Elizabeth Podowski, and their co-workers for their years of work in Lau Basin, making this study possible. Fanny Girard and Sam Vohsen provided valuable assistance, input, and feedback throughout the study. We thank Molly McGuigan and Alicia Yang for countless hours of help with digitizing and mosaicking. Arunima Sen, Verena Tunnicliffe, Vicki Ferrini, Roxanne Beinart, Peter Girguis, Meg Tivey, and Alison Swaddling shared their expertise and provided insightful comments. At sea activities were carried out with the approval of His Majesty's Cabinet of the Kingdom of Tonga (TN-085-2015 and a diplomatic note from the Ministry of Foreign Affairs and Trade of the Kingdom of Tonga F.7/2/3). This research was supported by the National Science Foundation under Grant Number NSF OCE 1537807.
References
Beaulieu, S. E. (2015). InterRidge Global Database of Active Submarine Hydrothermal Vent Fields: Prepared for InterRidge, version 3.3. [Online]. Available online at: http://vents-data.interridge.org
Boschen, R. E., Rowden, A. A., Clark, M. R., and Gardner, J. P. A. (2013). Mining of deep-sea seafloor massive sulfides: a review of the deposits, their benthic communities, impacts from mining, regulatory frameworks and management strategies. Ocean Coast. Manage. 84, 54–67. doi: 10.1016/j.ocecoaman.2013.07.005
Haymon, R. M., Fornari, D. J., Von Damm, K. L., Lilley, M. D., Perfit, M. R., Edmond, J. M., et al. (1993). Volcanic eruption of the mid-ocean ridge along the East Pacific rise crest at 9 45–52′ N: direct submersible observations of seafloor phenomena associated with an eruption event in April, 1991. Earth Planet. Sci. Lett. 119, 85–101. doi: 10.1016/0012-821X(93)90008-W
Shank, T. M., Fornari, D. J., Von Damm, K. L., Lilley, M. D., Haymon, R. M., and Lutz, R. A. (1998). Temporal and spatial patterns of biological community development at nascent deep-sea hydrothermal vents (9 50′ N, East Pacific Rise). Deep Sea Res. II 45, 465–515. doi: 10.1016/S0967-0645(97)00089-1
Δεν υπάρχουν σχόλια:
Δημοσίευση σχολίου
NO COMMENTS!